How NASA Will Use Robots to Create Rocket Fuel From Martian Soil
Engineers are building a prototype of a robotic factory that will create water, oxygen, and fuel on the surface of Mars

The Martians: This artist’s rendering shows excavating robots that may one day operate on Mars, long before humans ever set foot on the planet.
The year is 2038. After 18 months living and working on the surface of Mars, a crew of six explorers boards a deep-space transport rocket and leaves for Earth. No humans are staying behind, but work goes on without them: Autonomous robots will keep running a mining and chemical-synthesis plant they’d started years before this first crewed mission ever set foot on the planet. The plant produces water, oxygen, and rocket fuel using local resources, and it will methodically build up all the necessary supplies for the next Mars mission, set to arrive in another two years.
This robot factory isn’t science fiction: It’s being developed jointly by multiple teams across NASA. One of them is the Swamp Works Lab at NASA’s John F. Kennedy Space Center, in Florida, where I am a team lead. Officially, it’s known as an in situ resource utilization (ISRU) system, but we like to call it a dust-to-thrust factory, because it turns simple dust into rocket fuel. This technology will one day allow humans to live and work on Mars—and return to Earth to tell the story.
But why synthesize stuff on Mars instead of just shipping it there from Earth? NASA invokes the “gear-ratio problem.” By some estimates, to ship a single kilogram of fuel from Earth to Mars, today’s rockets need to burn 225 kilograms of fuel in transit—launching into low Earth orbit, shooting off toward Mars, slowing down to get into Mars orbit, and finally slowing to a safe landing on the surface of Mars. We’d start with 226 kg and end with 1 kg, which makes for a 226:1 gear ratio. And the ratio stays the same no matter what we ship. We would need 225 tons of fuel to send a ton of water, a ton of oxygen, or a ton of machinery. The only way to get around that harsh arithmetic is by making our water, oxygen, and fuel on-site.
Different research and engineering groups at NASA have been working on different parts of this problem. More recently, our Swamp Works team began integrating many separate working modules in order to demonstrate the entire closed-loop system. It’s still just a prototype, but it shows all the pieces that are necessary to make our dust-to-thrust factory a reality. And although the long-term plan is going to Mars, as an intermediate step NASA is focusing its attention on the moon. Most of the equipment will be tried out and fine-tuned on the lunar surface first, helping to reduce the risk over sending it all straight to Mars.
Dirt or dust on any heavenly body is commonly referred to as regolith. It’s most often just volcanic rock that has been crushed or weathered over time into a fine powder. On Mars, beneath a layer of rusting iron minerals that gives the planet its famous reddish hue, lies a thicker layer of silicates with names like feldspar, pyroxene, and olivine, which are made up of silicon and oxygen structures bonded to metals like iron, aluminum, and magnesium.
Excavating this material is challenging because its consistency and compactness varies from place to place on Mars. But what makes the task even harder is the planet’s low gravity, which makes it difficult to push a shovel into the ground without using your weight to counteract that force. On Earth, when we dig dirt we often use large vehicles because their heavy bodies react appropriately to the forces of the much smaller digging bucket. But remember the gear-ratio problem: Every ounce that we launch to Mars is precious and very costly. So we had to figure out a way to dig on the surface of Mars using very lightweight equipment.
aspect_ratioSpace Digger: NASA is developing a robotic excavator with two opposing bucket drums that can dip down and spin in opposite directions. This approach cancels out much of the digging forces, allowing the robot to operate in low gravity.Photo: Glen Benson/NASA
Enter RASSOR, or Regolith Advanced Surface Systems Operations Robot, an autonomous mining vehicle designed for one specific purpose: To dig or excavate regolith in low-gravity conditions. In designing RASSOR—pronounced “razor”—NASA engineers paid special attention to its actuation system. Robot actuators consist of motors, gearboxes, and other mechanisms that end up being a large percentage of the final mass of the system. In our design, we used frameless motors, electromagnetic brakes, and 3D-printed titanium housings, among other things, to minimize weight and volume. That effort paid off: Our design has about half the mass of current off-the-shelf actuators with similar specs.
To dig, RASSOR uses two opposing bucket drums, each outfitted with several small and toothy digging scoops. When RASSOR’s bucket drums spin and the arms that hold them dip down, they scrape up just a small amount of regolith into each digging scoop as it drives slowly forward. This creates a shallow slot trench rather than a deep hole. These rotating and digging bucket drums are hollow inside, allowing them to collect and hold the excavated regolith. Another key feature of RASSOR is that, while digging, the bucket drums actually spin in opposite directions. This cancels out much of the digging forces and will allow RASSOR to excavate in low gravity.
Once RASSOR has filled up its bucket drums, it raises its arms and drives to a processing facility. To unload the regolith, the robot simply spins the drums in reverse and the regolith pours out from the same small scoops that it entered. Another piece of equipment, a robotic hopper-lift arm, handles the next task. It hoists a load of regolith up to the deck of the factory and transfers it into an oven, which seals itself and starts to heat up. Any water molecules attached to the regolith are driven off by a dry gas blower, then collected using a condensation tube known as a cold finger.
You may be asking, “But isn’t the regolith on Mars bone-dry?” The answer is…it’s complicated. It depends mostly on where you dig, and how deep you dig. Some areas on Mars seem to have nearly solid sheets of water ice just a few feet below the surface. At the lower latitudes there are gypsum sand dunes consisting of about 8 percent water by weight.
Once stripped of its water, the regolith is dumped on the ground, so the RASSOR robot can scoop it up and haul it away. This “waste” material can actually be used for construction of protective structures or even roads and landing pads, using 3D-printing methods now under development at NASA.
1. MINING: A wheeled robot excavates regolith by spinning bucket drums outfitted with toothy digging scoops.
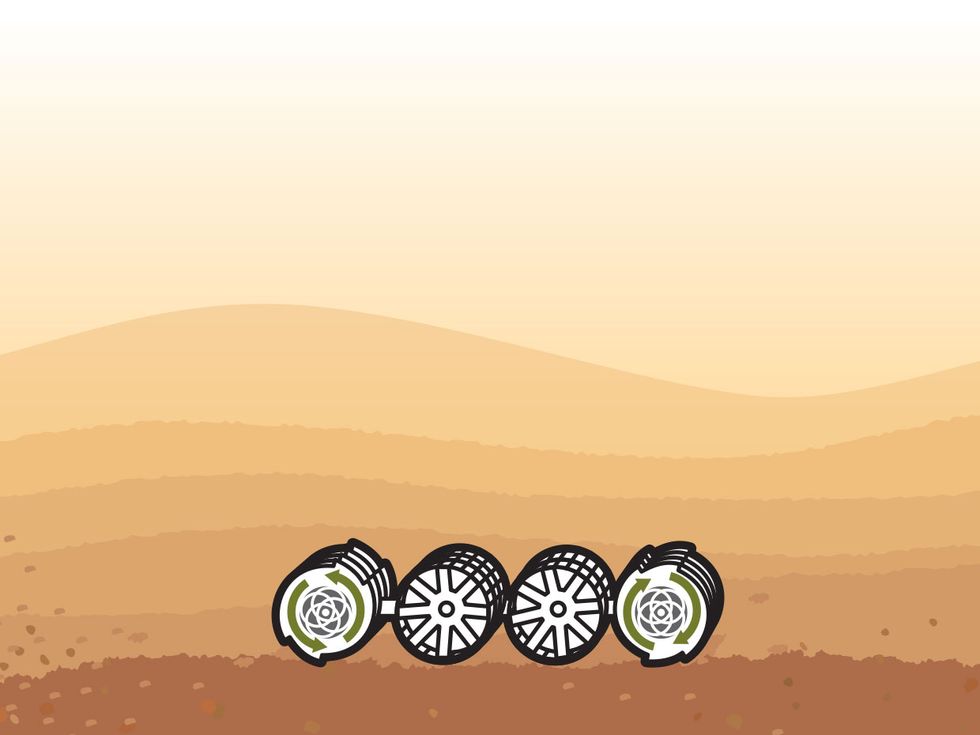
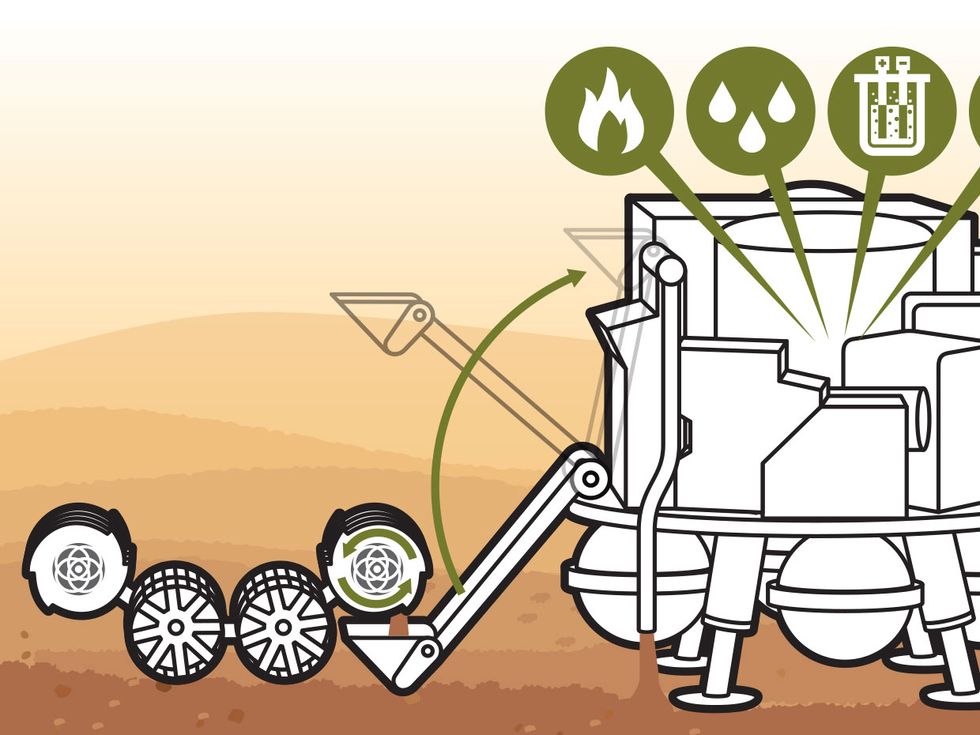
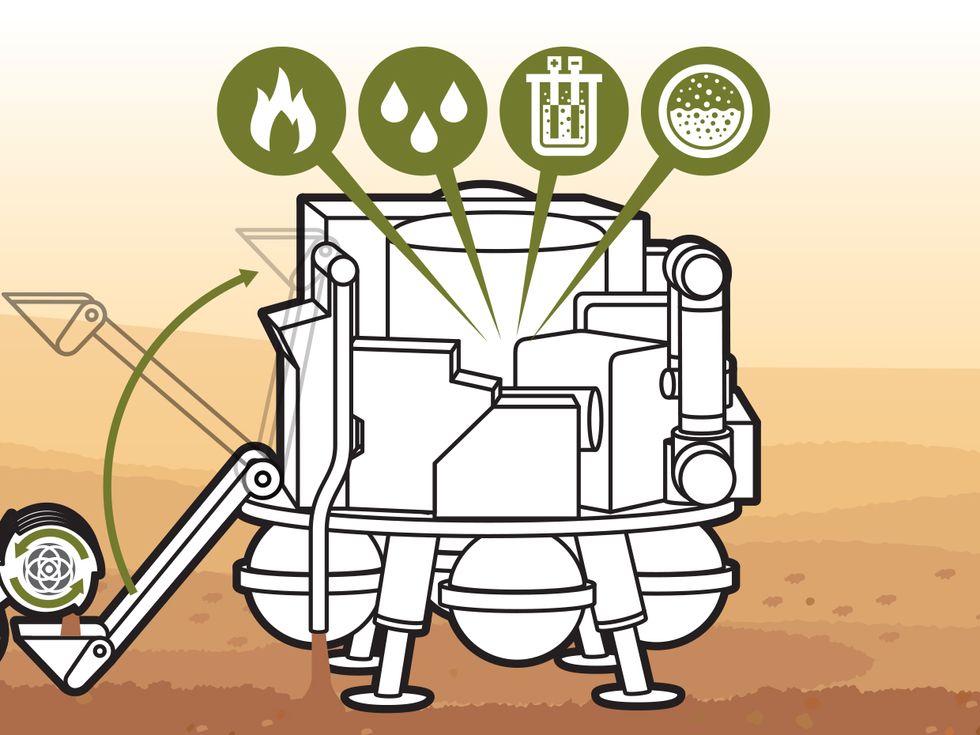
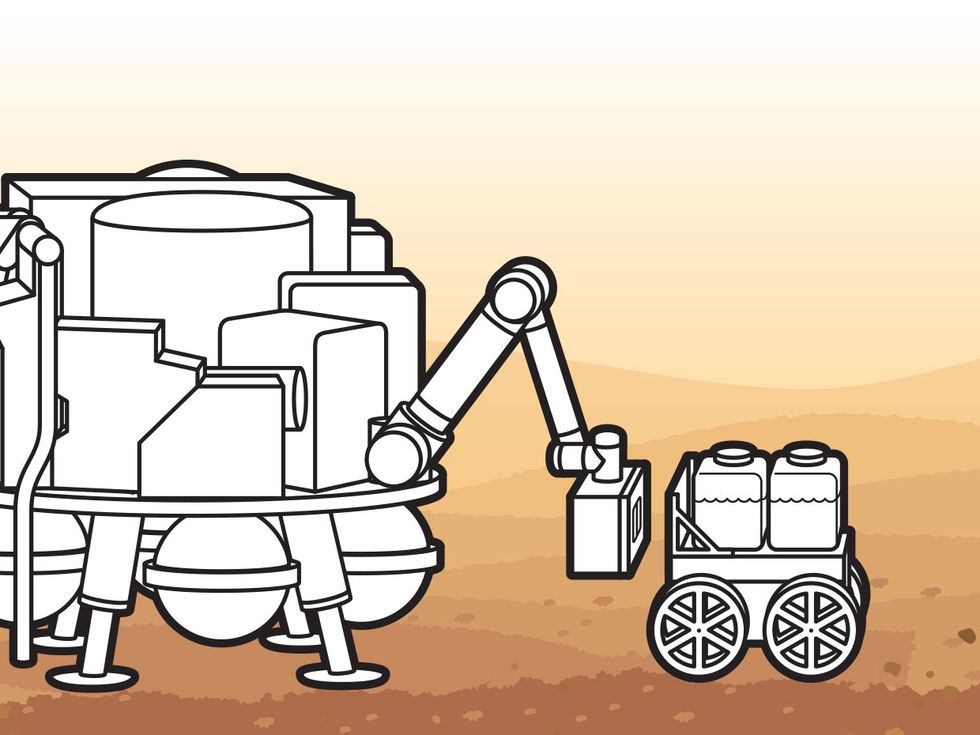
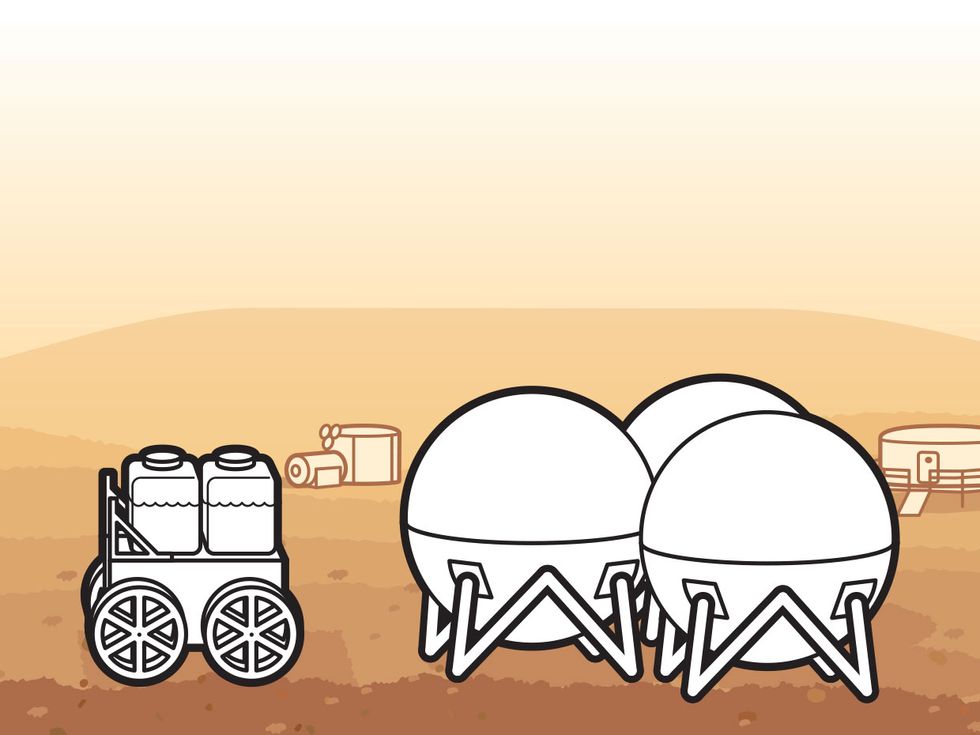
All the water that is pulled from the regolith is then purified. The cleanup module employs a multistage filtration system along with several deionizing beds.
The water isn’t just for drinking; it’s also the key ingredient for rocket fuel. By splitting the H2O molecules with an electrolyzer into hydrogen gas molecules (H2) and oxygen gas molecules (O2), and then compressing and liquefying both of those gases separately, we can synthesize the fuel and the oxidizer that are most commonly used in liquid-fueled rocket engines.
The problem is that liquid hydrogen must be stored at extremely low temperatures. That’s why NASA plans to turn the hydrogen into a type of fuel that is much easier to store: methane (CH4). You can get it by combining hydrogen with carbon. But where do we get the carbon on Mars?
Luckily, Mars has plenty of carbon. The Martian atmosphere is made up of 96 percent carbon dioxide gas molecules. Capturing that carbon is the job of a carbon dioxide freezer; it basically makes dry ice out of thin air.
Once we have collected hydrogen gas from the electrolyzer and carbon dioxide from the atmosphere, we can combine them into methane gas thanks to a chemical process called the Sabatier reaction. A special reactor NASA is designing creates the pressure and temperature needed to maintain that reaction and turn hydrogen and carbon dioxide into methane gas, with water as a by-product.
The next piece of equipment in our factory is a robotic umbilical arm for transferring fluids to an external tanker. What is unusual about this system is that its umbilical is specifically designed to keep out dust. Regolith dust is very fine, and it gets into everything. And because regolith is composed of crushed volcanic rock, it’s very abrasive and quite hard on equipment. (NASA’s moon missions showed that regolith was responsible for numerous problems, including false instrument readings, clogging of mechanisms, seal failures, and thermal control glitches.) So keeping it out of umbilicals, electrical and fluids connectors, and any sensitive electronics is critical.
Fill It Up: The author, Kurt W. Leucht, programs a robotic arm to connect a dust-tolerant umbilical to a mobile tanker robot. The umbilical is designed to fill the robot’s tanks with liquid fuel, water, and oxygen.Photos: Glen Benson/NASA
Each side of the umbilical contains a set of doors that acts sort of like an air lock, to keep out the dust. Completing a connection requires three separate steps: The first involves pressing the closed doors on each side together, so that a special perimeter seal creates a dustproof barrier completely around both sets of umbilical doors. In the second step, the doors on both sides of the umbilical interface open inside the dustproof seal, revealing the actual connectors, which are mounted on a moving plate. The final stage involves moving the plates together and physically mating all of the power, electrical, and fluids connectors.
A robotic arm onboard the rocket-fuel factory will pick up the umbilical and lower it to a mobile tanker robot, which will then connect and off-load the end products. In this respect, the surface-processing system is a lot like a filling station here on Earth, but instead of gasoline, it could dispense water. Or liquid oxygen. Or liquid methane. Or all three!
We recently demonstrated this ISRU factory at the Swamp Works Lab in Florida. At this point we had to simulate the oven and electrolyzer to reduce cost and complexity. We also simulated the three different end products by using water for all three. But we used working prototype hardware and software for all the other pieces.
By putting these subsystems together, we investigated some issues and failures along the way and learned several important lessons that we wouldn’t have learned until much later if we’d done our system integration only at the end of our development and testing process. This is one of the main tenets of Swamp Works: rapid prototyping and early integration, allowing quick proofs of concept and early failures.
The idea for this Mars rocket-fuel factory is that it will all be packed up into a neat little box, shipped to Mars, and deployed and started up on the Martian surface long before human explorers arrive. Human missions to Mars will depend on this factory to autonomously produce and store fuel for their return trip even before those astronauts launch from Earth. There are also teams at NASA figuring out how to grow all sorts of different stuff during transit and on Mars. Including potatoes.
So what needs to happen between now and then? Well, quite a lot.
NASA has years of experience with standalone landers and independent rovers operating on the surface of Mars. And our most recent rovers—Curiosity, which landed in 2012, and the Mars 2020 rover, to launch in 2020—do have a certain amount of autonomy built in. But the complexity of this Mars rocket-fuel factory, and the long runtime and level of autonomy that will be required of such a system, will take things to a whole new level.
Tons of Dust: To test its excavating robots, NASA uses an enclosed facility with more than 100 metric tons of crushed volcanic rock. The material serves as an analogue for the extremely fine and abrasive dirt particles found on the surface of Mars.Photo: Glen Benson/NASA
In the meantime, there are numerous technical challenges that we need to overcome before a mission like this can succeed. One of the most critical questions is whether each subsystem of our current Mars surface-processing system can scale up to meet the needs and throughput required by a human mission to Mars. Recent NASA studies estimate that a system like this will need to produce about 7 metric tons of liquid methane and about 22 metric tons of liquid oxygen in about 16 months. Then we must determine where to land and dig to maximize our yield, how many RASSOR excavators we’ll need, and how many hours per day they will have to operate. We also must work out how big the carbon dioxide freezer and Sabatier reactor need to be and how much power all this equipment will require.
How a Small-Town Kid Became a NASA Robotics Engineer
Photo: NASA
Photo: Kurt W. Leucht
When the fishing derby was over, the boy held up his catch and smiled at the camera. He didn’t win a prize, but it was a respectable haul. Especially for a kid who hated fishing.
Like other 10-year-olds growing up in Mackinaw, a small town tucked amid the cornfields of central Illinois, Kurt W. Leucht enjoyed the outdoors but had little patience for sitting and waiting for fish to bite. He also detested touching their slimy skin. What he loved was taking apart broken clocks and old telephones. And reading about space travel.
“My mom, who was a first-grade teacher, was the space shuttle’s biggest fan,” he says. “At least the biggest fan in town.”
Leucht is now a software engineer at NASA’s Kennedy Space Center (KSC), in Florida, far from the cornfields and fishing ponds of his childhood. How did a small-town kid end up building space robots for NASA? “Get comfortable,” he advises. “This will take a while.”
The short version: When Leucht was 13, his parents gave him an Apple IIe for Christmas. “That computer basically changed my life,” he says. He decided to become an engineer and later enrolled at Missouri University of Science & Technology, in Rolla. During college, he interned repeatedly at an electronics lab that was part of KSC, and after graduation he accepted a full-time job there.
In this issue, Leucht describes NASA’s plans for developing a robotic factory to turn Martian soil into water, oxygen, and fuel. “This technology,” he writes, “will one day allow humans to live and work on Mars—and return to Earth to tell the story.”
When that happens, the boy from Mackinaw will have played his part. And it won’t be just another fish story.
What’s more, we need to anticipate problems by identifying the potential single-point failures that could interrupt the surface-processing mission and thus delay the subsequent planned human mission. We’ll have to estimate the odds of each of those failures in order to add the right amount of duplication and redundancy to the system.
To make sure that the robotic technologies can support this mission for years on end without maintenance or repair, we’ll have to engineer them to very strict specifications. All moving parts need to keep out—or take the abuse from—those tiny little destructive regolith dust particles. Either beefing up the sealing technologies or beefing up the moving parts to tolerate the dust will add complexity and weight unless we can find some ingenious way around the problem.
We also need to find out how compact or hard the regolith and ice mixture is under the surface of Mars, and then design the digging implements appropriately. The current digging scoops and teeth on our RASSOR excavation robot work best on packed regolith mixed with chunks of ice. But this design won’t be appropriate for breaking up large sheets of tough solid ice. We will need definitive proof of the makeup of the ice and regolith beneath the surface on Mars in order to design the most appropriate and most efficient digging equipment. Either that, or we will have to design more complex and more robust tools that can handle a variety of soil densities and ice densities.
And we need to solve the challenges of long-term storage of supercold liquids. Pressure-vessel and insulation technologies and materials are constantly improving, but will current technologies work on the surface of Mars for long durations?
Over the next several years, NASA will investigate all of these challenges. And we will continue to increase the capabilities and readiness level of all of our prototype components. We will make the RASSOR robot stronger and lighter and test it in Mars-like environments. We will continue testing and integrating the oven and the electrolyzer, and we’ll attempt to scale up the carbon dioxide freezer and Sabatier reactor to verify that they can meet the needs of a crewed Mars mission. All of this work and more will continue forward so our dust-to-thrust prototype can one day become a fully operational system on Mars.
This article appears in the November 2018 print issue as “From Dust to Thrust.”